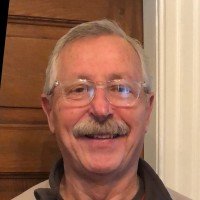
Robert Thomas : Principal Consultant at Scientific Solutions
Heavy Metals in Cannabis and Hemp
Cannabis and hemp are known to be hyper-accumulators of contaminants in the soil. That is why they have been used to clean up toxic waste sites where other kinds of remediation attempts have failed. In the aftermath of the Chernobyl nuclear melt down in the Ukraine in 1986, industrial hemp was planted to clean up the radioactive isotopes and heavy metals that had leaked into the soil and ground waters (2). Of course, Chernobyl is an extreme example of contamination, but as a result of normal human industrial activities over the past few decades including mining, smelting, electroplating, gasoline production, energy production, use of fertilizers, pesticides, waste treatment plants, paint and plumbing materials etc., heavy metal pollution has become one of the most serious environmental problems today. As a result, considerations about where cannabis and hemp are grown, is going to be critically important because it could have serious implications on the level of heavy metals that are being absorbed by the plant and as a result, the purity of cannabinoid consumer products that are used as therapeutics for patients with compromised immune. There are currently four heavy metals known as the big four (Pb, As, Cd, Hg), which are required by the thirty-six (36) states where medicinal and recreational cannabis is legal in the US. However, based on compelling evidence in the public domain, there are an additional ten (10) elemental contaminants found in natural ecosystems (soil, rocks, water, air) and from industrial anthropogenic activities that could be potential sources of contaminants accumulated by the plant. It’s only a matter of time before federal oversight will arrive and require the industry to expand this list of regulated elements beyond the big four. However, in the meantime, lead is getting the most attention by state regulators, not only because of its historical importance as a human toxicant, but also that elevated levels of lead have recently been found in many cannabis consumer products as reported in a recent ASTM workshop (3). Sources of Lead So, if lead represents the most serious ecological threat of all the heavy metal contaminants on the growing of cannabis and hemp, what are the most common environmental sources? There is no question that our historical dependence on using materials made from lead, including car batteries, paint pigments, gasoline, plumbing, ammunition, cable sheathing, lead crystal glass, radiation protection and solders has contributed to this problem. Decades of using these lead-based products are still having an impact on our soil and aquatic ecosystems and thus having a negative effect on the growing of cannabis and hemp. Some of these sources include:- The long-term weathering of galena rocks (lead sulfide), the most common of the lead-based ores will produce a lead-rich soil in the surrounding areas.
- Lead smelting plants and refineries generate copious amounts of lead dust and particulates, which gets into the environment and the soil.
- Automobile emission residues particularly from tetra ethyl lead used in the production of leaded gasoline before it was banned in 1996, is one of the major reasons for extremely high levels of lead in soil, particularly around major highways.
- Approximately 50 years of using lead-based paint before it was banned in 1978, particularly in older buildings has contributed to high levels of lead in household dust and particulates.
- The use of leaded water pipes has also contributed to higher lead levels in the municipal drinking water supplies if it is not chemically treated to reduce corrosion, as demonstrated by the Flint, MI drinking water crisis in 2014.
- Many fertilizers and nutrients used to grow cannabis and hemp are sourced from phosphate rocks, which are notorious for containing high levels of lead and other heavy metals.
- Many electronic cannabis delivery vaping systems have components made from brass, which often contains lead to make it easier to machine. In addition, battery terminal wires are also connected using lead-based solder.
Lead Toxicity
Lead has no known biological or physiological purpose in the human body, but is avidly absorbed into the system by ingestion, inhalation and to a lesser extent by skin absorption (4). Inorganic lead in submicron size particles in particular can be almost completely absorbed through the respiratory tract, whereas larger particles may be swallowed. The extent and rate of absorption of lead through the gastrointestinal tract depend on characteristics of the individual and on the nature of the medium ingested. It has been shown that children can absorb 40–50% of an oral dose of water-soluble lead compared to only 3–10% for adults (5). Young children are particularly susceptible, because of their playing and eating habits and typically have more hand-to-mouth activity than adults (6). Lead is absorbed more easily if there is a calcium/iron deficiency, or if the child has a high fat, inadequate mineral and/or low protein diet. When absorbed, lead is distributed within the body in three main areas – bones, blood and soft tissue. About 90% is distributed in the bones, while the majority of the rest gets absorbed into the bloodstream where it gets taken up by porphyrin molecules (complex nitrogen-containing organic compounds providing the foundation structure for hemoglobin) in the red blood cells (7). It is therefore clear that the repercussions and health risks are potentially enormous, if humans (especially young children) have a long-term exposure to high levels of lead. Health Effects Lead poisoning affects virtually every system in the body, and often occurs with no distinctive symptoms. It can damage the central nervous system, kidneys, and reproductive system and, at higher levels, can cause coma, convulsions, and even death. Even low levels of lead are harmful and are associated with lower intelligence, reduced brain development, decreased growth and impaired hearing (8). The level of lead in someone’s system is confirmed by a blood-lead test, which by today’s standards is considered elevated if it is in excess of 5 µg/dL (microgram per deciliter or 50 parts per billion (ppb) (9). However, the long-term effects of lead poisoning have not always been well- understood. In the early-mid 1960s, remedial action would be taken if a blood lead level (BLL) (or threshold level as it was known then) was in excess of 60 µg/dL. As investigators discovered more sensitive detection systems and designed better studies, the generally recognized level for lead toxicity has progressively shifted downward. In 1970 it was lowered to 40 µg/dL and by 1978 the level had been reduced to 30 µg/dL. In 1985 the CDC published a threshold level of 25 µg/dL, which they eventually lowered to10 µg/dL in 1991. It stayed at this level until it was reduced to 5 µg/dL in 2012. However, as our understanding of disease improves and measurement technology gets more refined, this level could be pushed even lower in the future (10). Figure 1 shows the trend in blood lead levels considered elevated by the Centers for Disease Control (CDC), since the mid-1960s.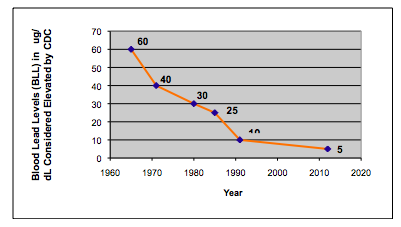
Routine Monitoring of Lead Using Atomic Spectroscopic Techniques
There is no question that the routine monitoring of has had a huge impact in reducing the number of children with elevated blood levels. Lead assays were initially carried out using the dithizone colorimetric method, which was sensitive enough, but very slow and labor intensive. It became a little more automated when anodic stripping voltammetry was developed (12), but blood-lead analysis was not considered a truly routine method until AS techniques became available. Let’s take a more detailed look at how improvements in atomic spectroscopy instrumentation detection capability have helped to lower the number of children with elevated blood lead levels, since atomic absorption was first commercialized in the early 1960s. Flame AA When FAA was first developed, the elevated level was 60 µg/dL. Even though this is equivalent to 600 parts per billion (ppb) of lead, which was well above the detection limit of ~20 ppb at the time, it struggled to meet this level when preparation and dilution of the blood samples was taken into consideration. This typically involved either dilution with a weak acid followed by centrifuging/filtering; or acid digestion followed by dilution and centrifuging/filtering. More recently dilution with a strong base like tetramethylammonium hydroxide (TMAH) and the addition of a surfactant to allow for easier aspiration has been used. When sample preparation was factored into the equation, the concentration of lead was reduced to 10-20 ppb – virtually the same as the FAA instrumental detection limit. Delves Cup AA To get around this limitation, an accessory called the Delves Cup was developed in the late 1960s to improve the detection limit of FAA (13). The Delves Cup approach uses a metal crucible or boat usually made from nickel or tantalum, which was positioned over the flame. The sample, typically 0.1-1.0 mL is pipetted into the cup, where the heated sample vapor is passed into a quartz tube, which was also heated by the flame. The ground sate atoms generated from the heated vapor are concentrated in the tube and therefore resident in the optical path for a longer period of time, resulting in much higher sensitivity and about 100x lower detection limits. The Delves Cup became the standard method for carrying out blood lead determinations for many years, because of its relative simplicity and low cost of operation. Unfortunately, the Delves Cup approach was found to be very operator dependent, not very reproducible (because of manual pipetting) and required calibration with blood matrix standards (14). It was still widely used but became less attractive with the commercialization of electrothermal atomization in the early-1970s. This new approach offered a detection capability for lead of ~ 0.1 ppb – approximately 200x better than FAA. However, its major benefit for the analysis of blood samples was the ability to dilute and inject the sample automatically into the graphite tube with very little off-line sample preparation. In addition, because the majority of the matrix components were “driven-off” prior to atomization at ~ 3000 °C, interferences were generally less than the Delves Cup, which only reached the temperature of the air/acetylene flame at ~2000 °C. This breakthrough meant that blood lead determinations, even at extremely low levels, could now be carried out in an automated fashion with relative ease. Zeeman Correction GFAA The next major milestone in AA was the development of Zeeman background correction (ZBGC) in 1981, which compensated for non-specific absorption and structured background produced by complex biological matrices, like blood and urine (15). This, in conjunction with the STPF (stabilized temperature platform furnace) concept, allowed for virtually interference-free graphite furnace analysis of blood samples, using aqueous calibrations (16). Such was the success of the ZBGC/STPF approach, due primarily to the fact that it could analyze many different kinds of samples using simple aqueous standards, that it became the recognized way of analyzing most types of complex matrices by GFAA. Even though GFAA had been the accepted way of doing blood lead determinations for over 15 years, the commercialization of quadrupole-based ICP-MS in 1983 gave analysts a tool that was not only 100x more sensitive but suffered from less severe matrix-induced interferences than GFFA. In addition, ICP-MS offered multielement capability and much higher sample throughput. These features made ICP-MS very attractive to the clinical community, such that many labs converted to ICP-MS as their main technique for trace element analysis. Then as the technique matured, utilizing advanced mass separation devices, performance enhancing tools, powerful interference reduction techniques and more flexible sampling accessories, detection limits in real-word samples improved dramatically for some elements. Figure 2 shows the improvement in detection capability (in ppb) of ICP-MS compared to ETA and the other AS techniques. It should also be emphasized these are instrument detection limits (IDLs), which are based on simplistic calculations of aqueous blanks carried out by manufacturers and not realistic method detection limit (MDL) or procedural limits of detection (PLOD) taking into consideration the sample preparation procedure, dilution steps and multiple analytical measurements (17). They are also only intended to be used as a guideline for comparison purposes because there are so many different ways of assessing detection capability, based on variations in manufacturer, instrument design and methodology.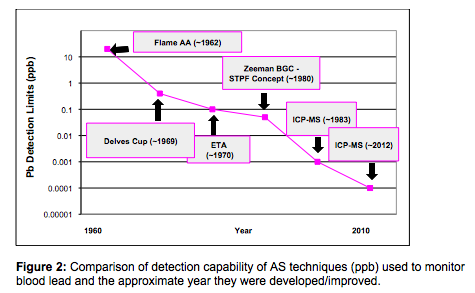
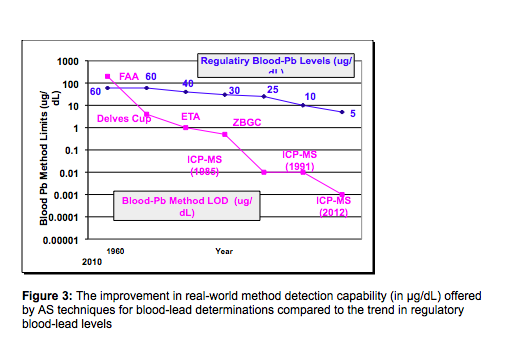
Final Thoughts
There is no question that developments in AS and ICP-MS instrumentation has helped us to better understand the toxicity effects of lead over the past 50 years. In particular, it has allowed us to lower the lead threshold level of 60 µL/dL in the mid-1960s to the current blood lead reference value of 5 µL/dL. More importantly, it has helped to reduce elevated blood levels of children in the U. S., from 26 % in the early-mid-1990s to less than 2% in 2014, as well as allowing us to get a much better understanding of the environmental sources of lead contamination. In particular, it has allowed us to be able to detect lead contaminant levels in cannabis and cannabis consumer products However, such is the power and versatility of modern atomic spectroscopy instrumentation and its accessories, that it has also dramatically improved our understanding of other trace metal-related human diseases. The toxic effects of trivalent/pentavalent arsenic and hexavalent chromium or the nutritional benefits of different selenium species would still be relatively unknown, if it wasn’t for the continual improvements in ICP-MS and in particular, its use as a very sensitive detector for trace element speciation studies using chromatographic separation technology. Even though ICP-MS has been successfully applied to many application areas since it was first commercialized in 1983, its use as a biomedical, clinical and toxicological research tool has had a direct impact on the quality of many people’s lives.Further Reading
- Elemental Impurities in Pharmaceuticals: Updates: United States Pharmacopeai (USP) Website: http://www.usp.org/chemical-medicines/elemental-impurities-updates
- From Laboratory Experiments to Large Scale Application – An Example of the Phytoremediation of Radionuclides, P. Soudek et.al. Advanced Science and Technology for Biological Decontamination of Sites Affected by Chemical and Radiological Nuclear Agents, pp 139-158, 2 007, https://www.researchgate.net/publication/226765625_FROM_LABORATORY_EXPERIMENTS_TO_LARGE_SCALE_APPLICATION__AN_EXAMPLE_OF_THE_PHYTOREMEDIATION_OF_RADIONUCLIDES
- A Recap of ASTM’s Workshop on Measuring Elemental Contaminants in Cannabis and Hemp Consumer Products, Robert Thomas, Analytical Cannabis, August 5, 2021, https://www.analyticalcannabis.com/articles/a-recap-of-astms-workshop-on-measuring-elemental-contaminants-in-cannabis-and-hemp-consumer-313229
- Savory and M. R. Willis, Clinical Chemistry, 40, 1387 (1994)
- Agency for Toxic Substances and Disease Registry (ATSDR), Toxicological Profile for Lead, Section 3.3: Toxicokinetics, August, 2007, (https://www.atsdr.cdc.gov/toxprofiles/TP.asp?id=96&tid=22).
- Preventing Lead Poisoning in Young Children, Chapter 2: Absorption of Lead, Centers for Disease Control and Prevention (CDC), 1991, https://www.cdc.gov/nceh/lead/publications/books/plpyc/contents.htm
- L. Needham, Case Studies in Environmental Medicine-Lead Toxicity, U. S. Dpt. of Health and Human Services (1990)
- Preventing Lead Poisoning in Young Children, Lead Information Page, Centers for Disease Control and Prevention (CDC), https://www.cdc.gov/nceh/lead/default.htm
- Childhood Blood Lead Levels in Children Aged <5 Years: United States, 2009–2014, Morbidity and Mortality Weekly Report (MMWR), Surveillance Summaries / January 20, 2017 / 66 (3);1–10, https://www.cdc.gov/mmwr/volumes/66/ss/ss6603a1.htm
- CDC Response to Advisory Committee on Childhood Lead Poisoning Prevention Recommendations in “Low Level Lead Exposure Harms Children: A Renewed Call of Primary Prevention” (2012) , https://www.cdc.gov/nceh/lead/ACCLPP/blood_lead_levels.htm
- Record of Proceedings from the Meeting of the Lead Poisoning Prevention Subcommittee of the NCEH/ATSDR Board of Scientific Counselors, Centers for Disease Control and Prevention (CDC), Atlanta, GA, September 19, 2016
- Centers for Disease Control and Prevention (CDC), Morbidity and Mortality Weekly Report (MMWR), October 7, 2016 / 65(39); 1089, Source: The National Health and Nutrition Examination Survey (NHANES); http://www.cdc.gov/nchs/nhanes/index.htm.
- Constantini, R. Giordano, M. Rubbing. Journal of Microchemistry, 35,70 (1987)
- T. Delves, Analyst, 95, 431 (1970)
- Cabet, J. M. Ottoway and G. S. Fell, Research and Development Topics in Analytical Chemistry, Proc. Analyt. Div. Chem. Soc., 300 (1977)
- Slavin, Sci. Total Environ., 71, 17 (1988)
- Quality Assurance of Chemical Measurements, 1st Edition, J. K. Taylor; CRC Press, Boca Raton, FL, ISBN 9780873710978, (1987)
- R. Jones et. al., Analysis of Whole Human Blood for Pb, Cd, Hg, Se, and Mn by ICP-DRC-MS for Biomonitoring and Acute Exposures. Talanta 162, 114–122, (2017), https://www.sciencedirect.com/science/article/pii/S0039914016307305